Graphene opens door to tunable transistors, LEDs
| 10 June 2009
BERKELEY — Today's transistors and light emitting diodes (LED) are based on silicon and gallium arsenide semiconductors, which have fixed electronic and optical properties.
Now, University of California, Berkeley, researchers have shown that a form of carbon called graphene has an electronic structure that can be controlled by an electrical field, an effect that can be exploited to make tunable electronic and photonic devices.
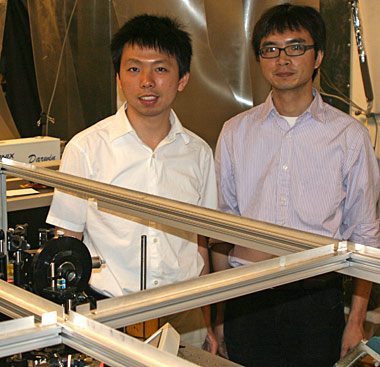
The bandgap of a material is the energy difference between electrons residing in the two most important states of a material - valence band states and conduction band states - and it determines the electrical and optical properties of the material.
"The real breakthrough in materials science is that for the first time you can use an electric field to close the bandgap and open the bandgap. No other material can do this, only bilayer graphene," Wang said.
Because tuning the bandgap of bilayer graphene can turn it from a metal into a semiconductor, a single millimeter-square sheet of bilayer graphene could potentially hold millions of differently tuned electronic devices that can be reconfigured at will, he said.
Wang, post-doctoral fellow Yuanbo Zhang, graduate student Tsung-Ta Tang and their UC Berkeley and Lawrence Berkeley National Laboratory (LBNL) colleagues report their success in the June 11 issue of Nature.
"The fundamental difference between a metal and a semiconductor is this bandgap, which allows us to create semiconducting devices," said coauthor Michael Crommie, UC Berkeley professor of physics. "The ability to simply put a material between two electrodes, apply an electric field and change the bandgap is a huge deal and a major advance in condensed matter physics, because it means that in a device configuration we can change the bandgap on the fly by sending an electrical signal to the material."
Graphene is a sheet of carbon atoms, each atom chemically bonded to its three neighbors to produce a hexagonal array that looks a lot like chicken wire. Since it was first isolated from graphite, the material in pencil lead, in 2004, it has been a hot topic of research, in part because solid state theory predicts unusual electronic properties, including a high electron mobility more than 10 times that of silicon.
However, the property that makes it a good conductor - its zero bandgap - also means that it's always on.
"To make any electronic device, like a transistor, you need to be able to turn it on or off," Zhang said. "But in graphene, though you have high electron mobility and you can modulate the conductance, you can't turn it off to make an effective transistor."
Semiconductors, for example, can be turned off because of a finite bandgap between the valence and conduction electron bands.
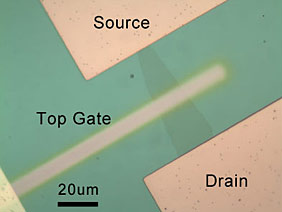
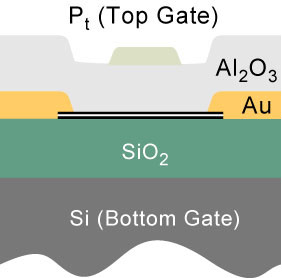
Wang, Zhang, Tang and their colleagues decided to construct bilayer graphene with two voltage gates instead of one. When the gate electrodes were attached to the top and bottom of the bilayer and electrical connections (a source and drain) made at the edges of the bilayer sheets, the researchers were able to open up and tune a bandgap merely by varying the gating voltages.
The team also showed that it can change another critical property of graphene, its Fermi energy, that is, the maximum energy of occupied electron states, which controls the electron density in the material.
"With top and bottom gates on bilayer graphene, you can independently control the two most important parameters in a semiconductor: You can change the electronic structure to vary the bandgap continuously, and independently control electron doping by varying the Fermi level," Wang said.
Because of charge impurities and defects in current devices, the graphene's electronic properties do not reflect the intrinsic graphene properties. Instead, the researchers took advantage of the optical properties of bandgap materials: If you shine light of just the right color on the material, valence electrons will absorb the light and jump over the bandgap.
In the case of graphene, the maximum bandgap the researchers could produce was 250 milli-electron volts (meV). (In comparison, the semiconductors germanium and silicon have about 740 and 1,200 meV bandgaps, respectively.) Putting the bilayer graphene in a high intensity infrared beam produced by LBNL's Advanced Light Source (ALS), the researchers saw absorption at the predicted bandgap energies, confirming its tunability.
Because the zero to 250 meV bandgap range allows graphene to be tuned continuously from a metal to a semiconductor, the researchers foresee turning a single sheet of bilayer graphene into a dynamic integrated electronic device with millions of gates deposited on the top and bottom.
"All you need is just a bunch of gates at all positions, and you can change any location to be either a metal or a semiconductor, that is, either a lead to conduct electrons or a transistor," Zhang said. "So basically, you don't fabricate any circuit to begin with, and then by applying gate voltages, you can achieve any circuit you want. This gives you extreme flexibility."
"That would be the dream in the future," Wang said.
Depending on the lithography technique used, the size of each gate could be much smaller than one micron - a millionth of a meter - allowing millions of separate electronic devices on a millimeter-square piece of bilayer graphene.
Wang and Zhang also foresee optical applications, because the zero-250 meV bandgap means graphene LEDs would emit frequencies anywhere in the far- to mid-infrared range. Ultimately, it could even be used for lasing materials generating light at frequencies from the terahertz to the infrared.
"It is very difficult to find materials that generate light in the infrared, not to mention a tunable light source," Wang said.
Crommie noted, too, that solid state physicists will have a field day studying the unusual properties of bilayer graphene. For one thing, electrons in monolayer graphene appear to behave as if they have no mass and move like particles of light - photons. In tunable bilayer graphene, the electrons suddenly act as if they have masses that vary with the bandgap.
"This is not just a technological advance, it also opens the door to some really new and potentially interesting physics," Crommie said.
Wang, Zhang, Tang and their colleagues continue to explore graphene's electronic properties and possible electronic devices.
Their coauthors are Crommie, Alex Zettl and Y. Ron Shen, UC Berkeley professors of physics; physics post-doctoral fellow Caglar Girit; and Zhao Hao and Michael C. Martin of LBNL's ALS Division. Zhang is a Miller Post-doctoral Fellow at UC Berkeley.
The work was supported by the U.S. Department of Energy.